Expanding the Possibilities: When and Where Can Grid-Enhancing Technologies, Distributed Energy Resources, and Microgrids Support the Grid of the Future?
This report discusses three categories of solutions that can bolster resilience, reliability, and affordability of electricity transmission: grid-enhancing technologies, distributed energy resources, and microgrids.
1. Introduction
Achieving the ambitious decarbonization goals established by the Biden administration, 100 percent clean electricity by 2035 and a net-zero emissions economy by 2050, requires substantially increasing the share of clean and renewable energy resources in the electricity generation mix. To reach these decarbonization targets cost-effectively, renewable power will need to grow to multiples of current levels. The transmission system is not equipped to handle the anticipated substantial increase in power flows; its lack of capacity and availability leads to grid congestion, which causes higher energy prices and curtails renewables. Moreover, with the anticipated electrification of the economy leading to large increases in future demand, the burden on the power grid is ever increasing. A report from the REPEAT project estimates that to take full advantage of the subsidies offered in the Inflation Reduction Act (IRA), transmission capacity must grow by about 2.3 percent per year, more than double the rate of the past 10 years (Jenkins et al. 2022).
Nevertheless, getting new projects built can take over a decade due to the complexities involved in their planning, siting, and permitting. State and federal policymakers have acknowledged this challenge and taken steps to support the buildout of new transmission. The Infrastructure Investment and Jobs Act (IIJA) and IRA carved out support for transmission expansion, allocating $12.5 billion and $5 billion, respectively. Furthermore, the Biden administration recently announced plans to streamline the federal permitting process for transmission lines through the Department of Energy (DOE) to facilitate buildout. Nonetheless, experts are still skeptical that we can build enough new transmission in time to support the shifting generation mix and increasing electrification to meet climate targets.
However, building new lines is not the only way to bolster resilience, reliability, and affordability. Several different types of investments can deliver similar outcomes. Grid-enhancing technologies can increase the capacity of existing lines, distributed energy resources can spread out generation resources so they are closer to load centers, and microgrids can use on-site power generation to support pockets of load and insulate campuses or communities from issues on the broader grid. These solutions can deliver benefits, but each is best suited to a specific set of circumstances and faces its own technical and regulatory barriers to implementation.
This report surveys the literature on different types of grid solutions. We discuss how the technologies work, in what circumstances they may act as substitutes for transmission expansion, evidence of their impact, and challenges (both technical and regulatory) in implementing them. New lines will undoubtedly be an important piece of the energy transition, but exploring these additional types of investments can help us understand where and when lower-cost and more rapidly deployed alternatives can provide solutions to some of our transmission woes.
2. Grid Necessities
The need for new transmission is driven by two core goals of grid operators: reliability and affordability. New lines can increase reliability by connecting more generation resources to the grid and linking entire regions with diverse types of resources. For example, a line that connects a region with ample solar power to one with ample wind power can help extend power availability when one of those resources is unavailable. New transmission can also deliver on affordability, especially in deregulated regions with competitive generation. By increasing the number of generators with access to a market, load-serving entities have a greater opportunity to find low-cost power sources as greater competition leads to lower prices.
For an investment to be considered a transmission “substitute” in a certain situation, it must deliver increased reliability and affordability to the region of interest. In some situations, the investment would not displace the need for new transmission but could postpone it. These investments can deliver benefits in the near term, something that is challenging with new lines. The investments we explore improve reliability and affordability in the following ways: (1) relieving line congestion, (2) connecting low-cost resources (especially renewables) to load, (3) minimizing energy losses, and (4) increasing flexibility and response speed to handle uncertainties in real time.
We discuss three broad categories of investments that can, in certain situations, substitute for new transmission: grid-enhancing technologies (GETs) are hardware and software that improve the grid’s efficiency and reliability; distributed energy resources (DERs) are small-scale, modular resources and technologies that generate and supply electricity at or near the place of use; and microgrids are localized energy systems that can generate, store, and distribute electricity autonomously to connected facilities, with or without being connected to the traditional, high-voltage grid.
3. Grid-Enhancing Technologies
GETs enhance the capacity of high-voltage lines and reduce congestion. We explore the value of three main types of GETs—dynamic line rating (DLR), flexible alternating current transmission system (FACTS), and topology optimization (TO). We review each technology and its specific benefits and limitations.
3.1. Dynamic Line Rating
The maximum current-carrying capacity of overhead lines (called “ampacity”) depends on temperature, line sag/tension, the construction material, and weather (IRENA 2020). Traditionally, transmission system operators (TSOs) have used static line ratings (SLRs) that rely on a set of conservative weather measurements, such as low wind speed, high solar radiation, and high temperature, to predetermine a fixed level of ampacity. These ratings often vary by season, referred to as “seasonal static ratings” (Tsuchida et al. 2021). In contrast, DLR systems actively vary line ratings, based on real-time monitoring of environmental factors, such as ambient temperature, wind speed and direction, and sunlight (IRENA 2020). Ambient adjusted ratings (AARs) appear in some regions and lie in between SLRs and DLRs in dynamicity. These devices use line-specific data and ambient air temperature data but do not monitor changes in real time or consider solar radiation, wind, or other factors that produce more accurate line ratings in close to real time (Figure 1; New York Power Authority n.d.). Recent FERC Orders 881 and 881a require transmission providers to adopt AARs for transmission lines by July of 2025 (Thappetaobula 2023).
Figure 1. Different Types of Line Ratings
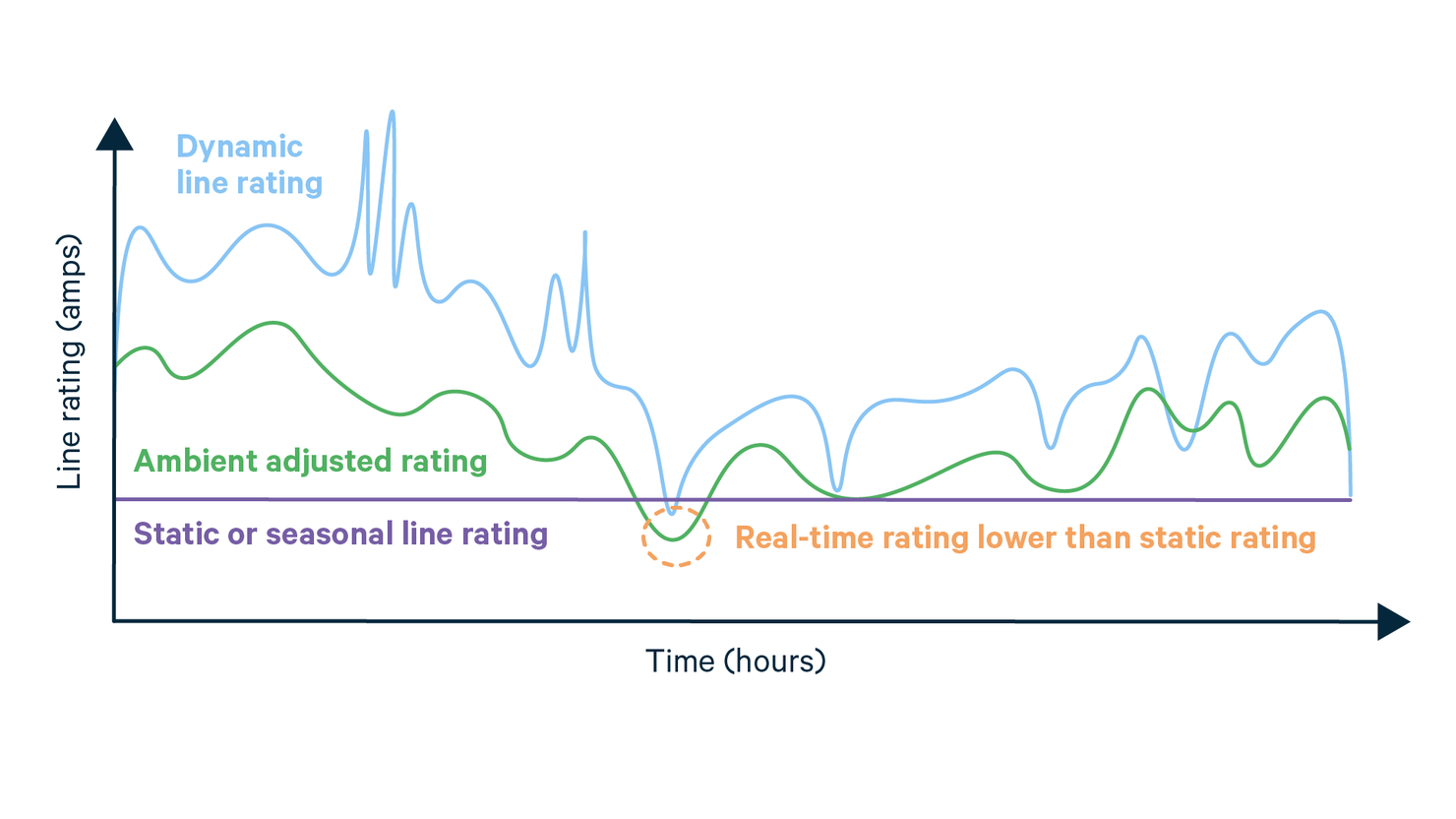
LR systems aim to optimize line use, thereby reducing congestion and improving the transfer capability of individual lines. To give a common transit analogy, SLR is like always keeping speed limits low to accommodate rainy and snowy road conditions, whereas DLR is like adjusting the speed limit in real time based on different weather and road conditions (Mendell et al. 2022; Tsuchida et al. 2021). In terms of physical infrastructure, a DLR system typically includes sensors on or near lines, information relaying communication and analytical systems that process DLR data, control systems, and operators who make decisions about line use based on the DLR information. Figure 2 depicts a DLR system (US DOE 2020).
Figure 2. A Conceptual DLR System
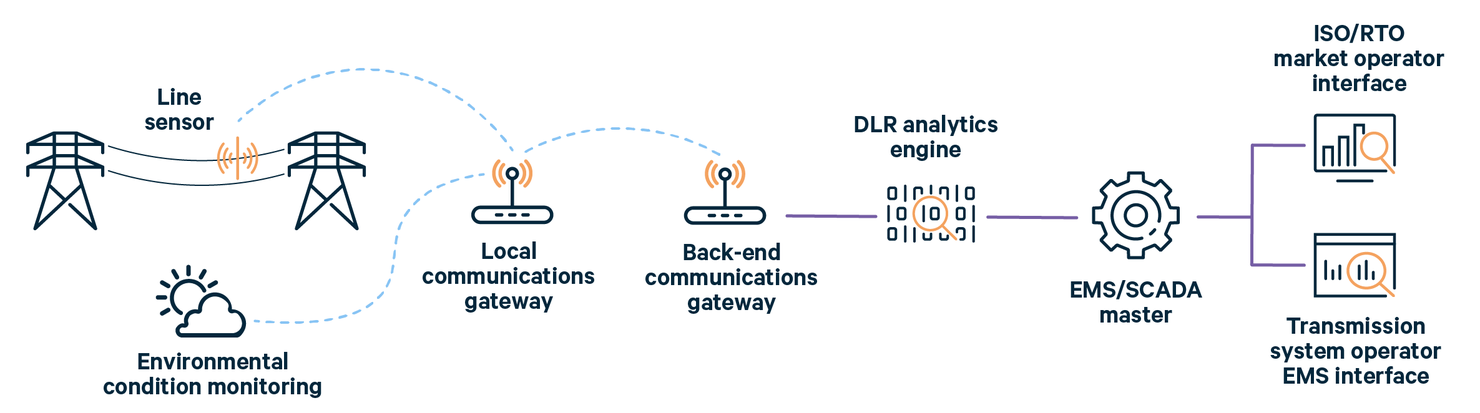
DLR systems could offer many benefits to the grid. A joint study by DOE and ONCOR found that DLRs can increase transfer capability of lines by 5–25 percent relative to SLR, which reduces congestion costs and renewables curtailment (Tsuchida et al. 2021). Capacity increases are especially pronounced for wind power integration because high winds simultaneously generate power and cool the lines nearby, increasing their transfer capacity (US DOE 2020). Two case studies in Pennsylvania and Upstate New York (Tsuchida et al. 2023) demonstrate how DLRs on congested lines would avoid $23.5 million in estimated annual congestion costs and reduce wind and solar curtailment by more than 350 MW.
DLR devices also have a lower cost and faster installation compared to reconductoring and rebuilding lines, which can take several years and require extended line outages. A PJM study showed that upgrading a line between Michigan and Illinois with DLR technology for $ 500,000 could save $4 million in annual congestion costs, whereas the traditional solution could cost $22–176 million (US DOE 2020). Furthermore, DLR devices can be deployed and relocated quickly, providing valuable flexibility to transmission owners (Tsuchida et al. 2023).
DLR technologies also protect the equipment from safety hazards by increasing situational awareness. For instance, real-time monitoring can inform operators when dynamic ratings fall below static ratings and lines are at risk, possible on a day with extreme heat and no wind. Regular data and feedback on lines help track the health of the system, detect any irregularities, and inform future forecasts for transmission and generation planning (US DOE 2020). DLRs can also enhance resilience when extreme weather events correlate with increased line-carrying capabilities, such as during a cold snap when demand for heating is abnormally high but lines can carry more current (US DOE 2020).
Although DLR systems have multiple benefits, they also have limitations. First, the device is prone to measurement and modeling errors that can result from improperly calibrated sensors, errors in weather forecasting and data collection, and sparse sensor deployment (US DOE 2020). Furthermore, DLRs are only effective on heavily loaded lines. Two DLR projects under DOE’s Smart Grid Demonstration Program found that the sensors cannot gather precise data for lightly loaded lines (Wang and Pinter 2014). Using DLRs on heavily trafficked lines and including confidence intervals in DLR estimates can mitigate the impact of errors.
Although ISOs and RTOs can leverage AAR within their existing control systems, incorporating DLR may require changing market operations or investing in new control systems, which can be expensive. The incremental benefits of DLR beyond AAR may be small in certain areas where wind speeds do not vary significantly. DLR projects may face another disadvantage from the incentives created by the structure of utility rate regulation. Specifically, DLRs are not capital intensive and thus contribute little to a utility’s ability to earn a rate of return, unlike investments in new lines and towers. Thus, owners’ current business models tend to favor capital-intensive approaches.
Overall, DLR is a low-cost technology to increase existing line capacity and provide congestion relief in the near term. It adds the most transfer capacity when the weather is cool, so it is likely better suited to a context with plentiful wind or congestion during winter peaks rather than congestion driven by high solar generation in a heat wave. Additionally, it does require lines that are more heavily loaded to function effectively. It would be most useful as a substitute for transmission in places with many existing lines with heavy loads and where new infrastructure for increasing capacity is challenging to build because of space constraints, such as densely populated areas.
3.2. Flexible Alternating Current Transmission System
Although DLR responds to a line’s thermal properties that limit the flow of current on individual circuits, often, these limitations are broader and related to overall network performance. Adding devices that provide dynamic voltage support and stability through enhanced system damping Damping is the ability to absorb or prevent oscillations from disturbances such as changes in load, faults, or line switching, which can cause blackouts and damage equipment due to large swings in voltage and frequency. can help overcome such issues. FACTS is an advanced power flow control system that uses static devices to pull and push power on lines to maximize their use, thus increasing the total current delivered. It enables fast, dynamic, and flexible control of power flow and voltage stability, without changing generator dispatch (US DOE 2020). Lines that have spare capacity can pull more power, reducing the constraint on another line and increasing the grid’s overall power transfer capacity (Tsuchida et al. 2021). Using the transit analogy, FACTS is akin to switching railroad tracks to redirect trains to available railway platforms when one platform is becoming constrained by too many train arrivals and departures (Mendell et al. 2022). Before more advanced FACTS devices were introduced in the 1970s, phase shifting transformers (PSTs) or phase angle regulators were used to perform similar functions, and they are still common. However, these first-generation mechanically switched devices have slow response characteristics. With the advancement and incorporation of solid-state switches and semiconductors, thyristor-controlled FACTS devices came about; these were faster and more robust. The latest-generation devices, such as the static synchronous compensator (STATCOM), static synchronous series compensator (SSSC), and unified power flow controller (UPFC), are all examples of FACTs that have fully controlled power semiconductors and provide greater flexibility and concurrent control over all the basic system parameters. However, their deployment is low due to their costs (US DOE 2020). Detailed information on the different kinds of FACTS technologies can be found in Singh and Agnihotri (2018), Okeke and Zaher (2013), Eslami et al. (2012), and Georgilakis and Vernados (2011).
FACTS offer several unique benefits. Advanced FACTS, especially the latest-generation devices, enhance response speed and flexibility to address disturbances, faults, and unforeseen situations destabilizing the grid As opposed to the aforementioned mechanical devices or no devices. (US DOE 2020). All such features can improve long-term grid reliability and resiliency. These devices are especially important for integrating intermittent renewables in the grid, as their variable nature can affect the voltage and frequency of power carried on the lines. Additionally, FACTS devices can reduce impact during line construction and upgrades. For example, one study found that installing FACTS devices on nearby lines to avoid overloading them during upgrades of other lines could save an estimated $70 million in avoided costs of redispatch over the 3.5 years of upgradation time. Moreover, the investment costs of installation would equal 2–6 percent of the avoided congestion costs (Tsuchida et al. 2023).
FACTS also faces limitations and barriers. The initial costs are quite high, and the devices require regular maintenance, which adds to the costs. Dynamic response FACTS technologies, such as SSSC, STATCOM, and UPFC, cost much more than PSTs without that ability. Nonetheless, they may still present significant savings over new transmission. Additionally, power flow control (PFC) technologies, in general, do not well suit underground line systems due to the space restrictions (US DOE 2020).
FACTS are also difficult to integrate into grid reliability planning. System planners consider worst-case scenarios, such as line outages, within their established planning models to determine whether the system can hold up. In interconnected networks, voltage and frequency constraint risks can be mitigated by shifting loads to other lines using FACTS devices. Transmission planners need to know how to model the impacts of this technology on the likelihood of outages rather than test its effectiveness when outages are exogenously modeled (US DOE 2020). More sophisticated methods and tools for modeling are needed to obtain a complete understanding of the impacts on stability and voltage limits. If planners do not consider the impacts of FACTS on reducing outages during planning, they will undervalue it.
FACTS may offer benefits to the broader grid, but a third party needs owners’ consent for any PFC upgrades. Additionally, FERC Order 1000 maintained the rights of incumbent owners with regards to owning, building, and recovering the costs of upgrades on their facilities and allows them to refuse such upgrades. This provision could potentially block the entry of new participants who want to sell PFC solutions to a competitive market. Another possibility is that if incumbents propose PFC upgrades as a way to relieve demand for new transmission, nonincumbents could see this as restricting construction of new transmission projects and thus restricting competition (US DOE 2020).
Overall, FACTS solutions are well suited for places that need rapid dynamic responses, have frequent load and voltage variation, and have fewer space restrictions to retrofit these relatively large physical assets (compared to other GETs). Thus, although DLR systems may serve better in densely populated areas, FACTS may be better for areas with more variable renewables generation and where generation needs to be efficiently interconnected to the grid, without lapses. Often, utility-scale renewable electricity generation happens in low-population areas, where space is plentiful, and where lines are built above ground. In such areas, FACTS could prove useful and cost-effective in increasing existing lines’ capacity while maintaining voltage and stability limits, reducing the need for new transmission to integrate renewable generation.
3.3. Topology Optimization
Topology in the power grid is how the branch elements, such as lines and transformers, are arranged for power supply. TO (an application of topology control) refers to software solutions that use artificial intelligence to identify ways to reconfigure the grid by rerouting power flows around bottlenecks, reducing congestion and optimizing transmission capabilities (US DOE 2020) by opening and closing circuit breakers to divert power from congested lines to other lines. Topology control algorithms (TCA), the primary TO tool, automatically identify the best configuration options to manage congestion, respond to contingencies, such as overloads and constraints, and help with planning outages. This software is like “Google Maps” for the grid, which assesses and presents the most viable route to take (Tsuchida et al. 2021; Ruiz 2017). Using the transit analogy, TO is akin to diverting traffic to less-congested roads to make traffic flow more smoothly on the congested ones (Mendell et al. 2022; Tsuchida et al. 2021). TC works similarly to hardware-based FACTS devices and can complement them. TO software can be used with existing equipment because the switching infrastructure is already in place and circuit breakers are controlled remotely over the supervisory control and data acquisition systems (Tsuchida et al. 2021). TSOs perform topology control on an ad hoc basis, relying heavily on operator experience (Figure 3). This manual process is becoming increasingly time-consuming given the growing complexity of the grid. Recent advances in artificial intelligence and the support of the Department of Energy Advanced Research Projects Agency—Energy (DOE ARPA—E) in developing TCAs have automated this process of finding optimal transmission control actions (US DOE 2020; Ruiz 2017).
Figure 3. How Transmission Topology Optimization Works
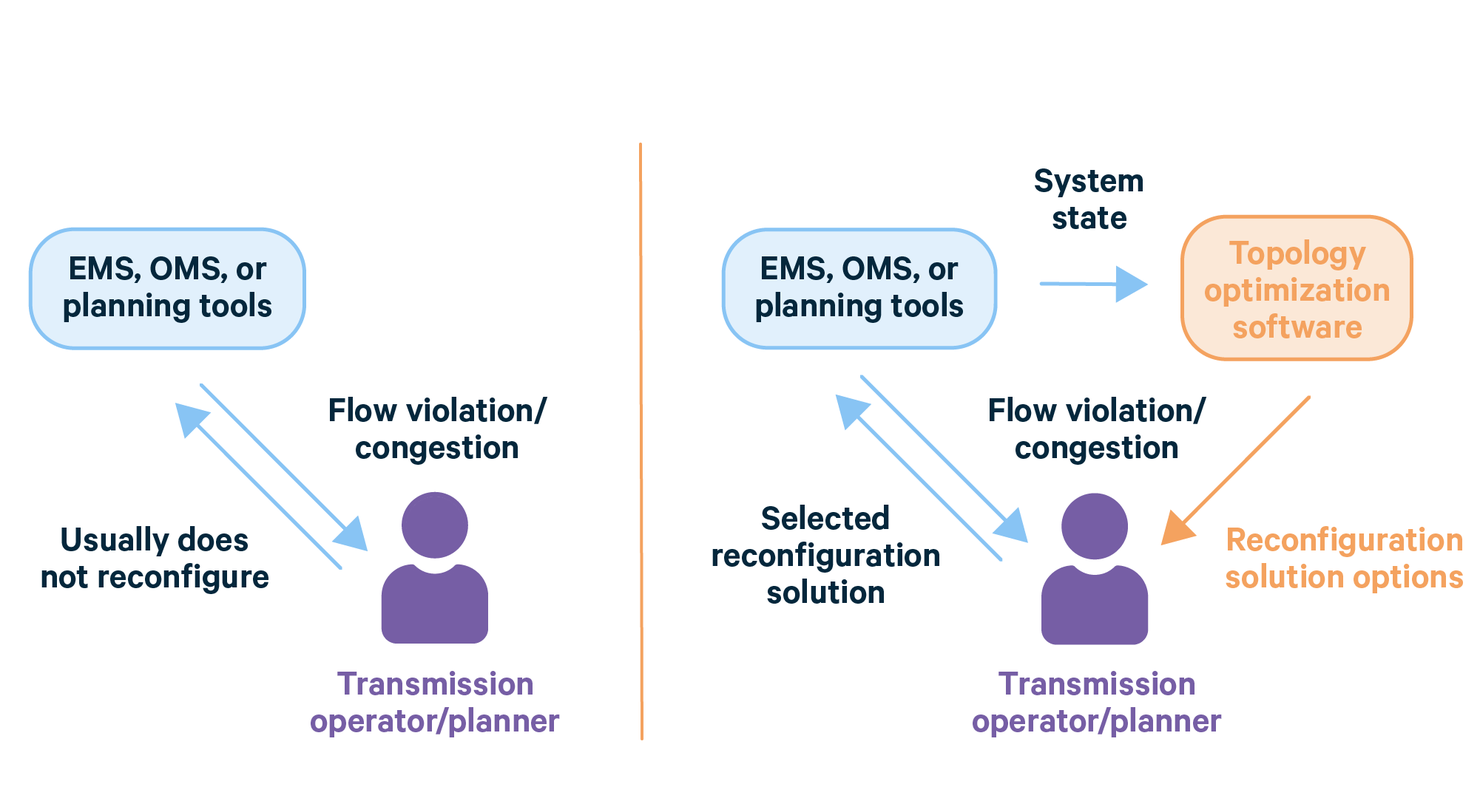
These TCAs have several benefits relative to manual reconfiguration, such as lower implementation costs, real-time solutions, and better management and planning. TCAs are designed to operate with existing systems and do not require capital investments or hardware installations, making them lower cost than other GETs, such as FACTS. According to a 2021 Brattle Group study (Tsuchida et al. 2021), the cost per switching cycle (opening and closing a circuit breaker) would be $10–100, which is a fraction of the congestion savings. Hence, in certain situations, TO may also prove to be a more cost-effective option than building new lines.
TCAs also quickly provide multiple options to the system operator along with an impact evaluation for each, which is especially useful for meeting contingency constraints and voltage criteria. Case studies in two RTOs—Southwest Power Pool (SPP) and Midcontinent Independent System Operator (MISO)—show that TO software found reconfiguration solutions for lines and transformers facing binding constraints, which increased flows reliably, thereby providing savings from avoided congestion costs (Tsuchida et al. 2023). In PJM, reconfigurations identified by TO software led to a 50 percent decline in real-time congestion costs, with an estimated value of approximately $100 million per year across the PJM footprint (US DOE 2020; Ruiz 2017). Furthermore, TO can reduce renewables curtailment, resulting in greater use of existing renewables, energy price reductions, and emissions benefits. In the SPP pilot of TO, congestion was curtailing 285 MW of wind, but TO relieved congestion and eliminated curtailment (US DOE 2020).
TO also improves operations management, reducing the number of system violations and outages. If a natural disaster alters the state of the lines, optimal corrective actions given by the TCAs can accelerate the recovery while keeping customer interruptions to a minimum, increasing overall system resilience. Additionally, TO improves operations planning by finding reconfiguration solutions for scheduled outages and real-time solutions for any system disruption occurring during them (US DOE 2020).
TO solutions are not effective in all cases. Due to the size of the US power grids, reconfiguring branches can become computationally intense. Moreover, because solutions need to be adopted in real time, the large number of possible line-switching combinations may make computing and identifying optimal solutions slow and laborious for operators (US DOE 2020). Reducing complexity by oversimplification is one solution but risks harming the results’ accuracy and reliability. Thus, more research on improving computational performance is needed, particularly as the grid continues to expand.
TO can also wear on the grid itself. Frequent switching of circuit breakers can expedite their aging, add to maintenance costs, and negatively impact reliability. Incorporating parameters, such as switching rates, into the TO problem and quantifying the costs of increased switching can help reduce these concerns. Moreover, constant breaker switching for TO could hamper grid stability and result in failures or blackouts. This concern is especially important as more variable and inverter-based renewables, such as batteries, wind, and solar, are deployed. Conventional controls may not be tuned to handle contingencies arising from these resources, and tuning them may not be that easy (US DOE 2020).
Day-ahead energy markets that predict next day’s loads and dispatch also include forecasts of local price differences due to line transmission limits, creating revenues for financial transmission right holders from a share of the congestion charges. As TO affects which lines are congested and reconfigures them in real time, it can impact day-ahead market structures and lead to financial losses if market participants do not model and factor in these dynamic changes (including potential transmission outages from switching actions) (US DOE 2020). These limitations can become barriers to adoption of this technology.
Overall, TO has an important cost advantage relative to FACTS, as it does not require physical capital investments. Thus, it complements capital-intensive PFC technologies, such as FACTS, by helping operators figure out the best ways to change power flows and optimize the transmission system. FACTS may also complement the TO software on subgrids where the share of intermittent renewables is high and could lead to grid destabilization. Last, with improvements in the software’s computational speed and ability, TO can support future investments in transmission upgrades by increasing lines’ long-term value and also defer line upgrades in the near term. In terms of relieving the need for new transmission, TO may be most impactful in areas with high renewables curtailment due to transmission congestion.
3.4. Key Findings on GETs
GETs maximize the capacity of existing rights of way, reducing the need for some new transmission projects and deferring the construction of others. Overall, these technologies are much less costly than new buildouts and have faster cost recovery potential (Tsuchida et al. 2023). They increase the flexibility of the existing system by providing multiple real-time power flow solutions based on the changing dynamics of the variables that affect the system. This flexibility is particularly important for economic efficiency as more intermittent, nondispatchable renewables penetrate the grid and changes in power flows need to occur much faster (US DOE 2020). Many GETs are scalable, can be relocated to other lines due to their portability, and can even be removed and reinstalled, unlike capital-intensive investments. While lines are built or upgraded, GETs can minimize outages’ frequency and length by optimizing grid reconfiguration. As new projects come into service, GETs can enhance their value, increasing the benefit–cost ratio of these traditional investments and potentially improving their approval rate (Tsuchida et al. 2023).
However, for all three GETs, the electricity markets and regulatory structures provide insufficient incentives to enable wide uptake and fast implementation (US DOE 2020). For example, control systems would need expensive updates to system operating software to integrate all the real-time data received from sensors and hardware devices. Dynamic systems enabled by advanced technologies would also add complexity to wholesale market operations, such as forecasting and bidding (US DOE 2020). Beyond the added system complexity, the regulatory structure incentivizes large capital investments upon which transmission owners receive a fixed rate of return. GETs usually do not involve large capital investments, especially compared to new transmission projects (Watt Coalition, n.d.). Moreover, the economic benefits of reduced congestion and using low-cost renewables accrue not to owners but to customers directly (US DOE 2020). As a result, the agents capable of implementing GETs have little incentive to do so. FERC order 2023 requires transmission providers to investigate alternative transmission technologies in their interconnection cluster studies, but it doesn’t force them to implement specific solutions (FERC 2023).
4. Distributed Energy Resources
DERs are small-scale electricity generation or storage sources that are located near where the electricity is needed. DERs include small residential, community, and commercial sources attached to the distribution grid. Distributed generators can be both renewable and nonrenewable. Distributed renewables are described in Cleary and Palmer (2022). Usually, less than 10 MW in capacity, DERs have rapidly expanded in the past 10–15 years; some estimates anticipate that capacity will double between 2022 and 2027 (Wood Mackenzie 2023). DERs are broadly categorized into fuel-based generation, zero-emissions generation, battery energy storage systems, demand response, and demand management through virtual power plants (VPPs). We discuss each of these and their specific benefits and limitations next.
4.1. Fuel-Based Distributed Generation
Fuel-based DERs include backup power generators, microturbines, biomass combustion, and combined heat and power systems. Typically, these provide essential backup power in cases of emergencies, outages, voltage issues from the grid, and severe weather events. Biomass combustion is a renewable DER; organic materials, such as agricultural and plant residues, wood, and animal waste, are converted to electricity using different methods, including direct burning or combustion (EIA 2023). All fuel-based DERs can be deployed at a commercial or industrial scale to meet demand at the customer site, especially during peak-load operations, and supply electricity to the distribution network.
Combustion-based DERs release heat while generating electricity. This thermal energy can be used for heating/cooling water or interior spaces and in industrial processes instead of burning more fuel. A process where both electric and thermal energy are used is called “combined heat and power” (CHP) or “cogeneration.” CHP can lower costs and environmental impacts of using fuel exclusively for heating and other purposes. Although fuel-based DERs provide reliability and low-cost options for DG, the clean electricity transition and decarbonization goals require eventually phasing out fossil DERs; these should be thought of as not a substitute for transmission but rather a source of potential demand increase as fossil fuels phase out. Users of fuel-based distributed generation will either turn to the grid (increasing strain on transmission networks) or transition to emissions-free distributed generation technologies.
4.2. Emissions-Free Distributed Generation
DERs that generate zero CO2 emissions include small-scale solar, wind, hydropower, and hydrogen fuel cells. The most common source is solar photovoltaic (PV) panels or solar arrays (connected panels) containing cells that convert sunlight directly into power. Small-scale solar installations are defined by EIA as those that produce less than 1 MW (Lee and Moses 2015). These PV systems are generally found on residential and commercial rooftops and typically average 5 kW One residential rooftop panel produces 250–400 Watts (Allen and Tynan 2023). and 200 kW, respectively (Mayes 2017). For community solar, off-site arrays serve multiple customers, such as homes and businesses. Solar panels are one of the fastest-growing renewable energy technologies due to declining costs and policy incentives. Feed-in tariffs (utilities purchasing solar power from homeowners and businesses at fixed rates) and net-metering (customers getting credits on the bill for surplus electricity sent to the grid) both support the growth of distributed solar (Cummins Inc. 2021). However, because of economies of scale, the levelized cost of energy (LCOE) LCOE is the net present value of the total cost of electricity generation of a power plant over an assumed lifetime. from utility-scale solar is 25–40 percent that of distributed solar, and NREL predicts that it will continue to be so in the future. Even by 2050, under the advanced technology scenario, the LCOE of residential PV is projected to be at least three times more than utility-scale PV, and commercial PV’s LCOE will be about 2.2 times higher. It is not clear how much of this cost gap is offset by the additional need for transmission and distribution lines or the potential environmental costs of centralized utility-scale generation. A 2015 study by the Brattle Group that takes such transmission and distribution costs into consideration concludes that utility-scale solar would still be cheaper (Tsuchida et al. 2015), but the analysis is limited to one utility and region of the country, and thus more research is needed.
Wind energy has the largest share of clean and renewable electricity in the United States, and grid-scale wind energy has grown at by about 30 percent per year over the last decade (EERE n.d.a). Distributed wind (DW) is defined by a plant’s proximity to end-use or distribution infrastructure and not its size. However, typically, DW energy uses smaller wind turbines that range from 5 kW to multi-MW and can be installed at the residential, agricultural, and small commercial and industrial scales (EERE n.d.b). DW is either installed on the “customer” side of the meter (e.g., a manufacturing facility meeting its own load) or to directly supply and support the distribution network. Residential turbines are 1–10 kW, whereas community-scale energy facilities have multi-MW turbines with total capacities up to 20 MW (EERE n.d.b). Like solar, the cost differences between distributed and utility-scale wind are large, especially for residential DW (6 times higher). However, by 2035 and 2050, the LCOE for commercial, midsize, and large DW is projected to be about 1.6–1.8 times that of land-based utility-scale wind, which may make these types of DW projects comparable and even cheaper, when including the transmission costs associated with utility-scale projects . The cost gap for residential DW is also expected to narrow to 2.1–2.4 times that of utility-scale land-based wind. Another zero-emissions DER is small-scale or micro hydropower generation. Hydroelectricity is the second-largest renewable electricity source in the United States. Although utility-scale plants (capacity of more than 30 MW) typically employ dams, small run-of-the-river turbines (up to 5 MW) can serve as DERs. The micro plants have a capacity up to 100 kW and may be built where rivers, streams, and other moving water sources are available, generating enough power for a single home or community (EERE n.d.c). Compared to hydropower dams, smaller run-of-the-river units have reduced environmental impact, and projects may be approved faster. Small-scale hydropower is generally dispatchable but can be impacted by droughts.
Last, a hydrogen fuel cell extracts hydrogen through a low- or zero-emitting thermochemical process and produces DC power. Hydrogen is fed (either directly or by reforming hydrogen-rich fuels, such as methanol and ethanol) to the anode (negative electrode) and air to the cathode (positive electrode) in an electrolyte bath, which produces electricity (EERE, n.d.d). The by-products of this process are water, heat, and some CO2 (when pure hydrogen is not used). Fuel cells are quiet during operation and therefore good for distributed generation at or near residential and commercial places (FEMP 2002). Additionally, reversible fuel cells, under development, can store excess electricity generated by solar and wind in the form of hydrogen (EERE n.d.e). However, fuel cells are more costly than other distributed energy sources, and research is investigating what kinds of electrolytes could be used to make them more efficient. In addition, the process of creating hydrogen from fuels such as natural gas or propane would produce emissions, so their ability to deliver zero-emissions power is dependent on an abundance of renewables (Bartlett and Krupnick 2020).
Overall, the zero-emission DERs described may only partially displace the need for transmission expansion in a renewables-dependent grid. In particular, both distributed solar and wind power produce clean electricity close to end users and can reduce the need for lines. However, both are variable sources, so customers still need to be connected to the grid to maintain reliable supply. To the extent a region relies heavily on one distributed resource type , the grid may experience large surges in demand when that resource is unavailable, increasing transmission strain. Additional investments, such as demand management and on-site storage, can help address this problem. Ultimately, factors such as population density, geography, and resource availability will determine whether higher LCOE of DERs are offset by the siting, permitting, and buildout costs of new transmission needed for transporting power from centralized generation. More research that captures the full customer and system benefits of distributed generation, such as demand-side management, impacts on customer bills, benefits to disadvantaged communities, and resiliency with extreme weather events, is needed to determine how well DERs can reduce the need for new transmission.
4.3. Battery Energy Storage
Energy storage devices capture energy from the grid or other sources, such as renewable DERs, and make it available during times of unexpected high demand, weather-related outages, or lack of wind and sunlight. Lithium-ion batteries, such as those used to power cellphones, laptops, and electric vehicles (EVs), are most common. Other types of batteries are being developed that would increase efficiency, storage duration, and affordability. The systems exist at various scales—from large centralized systems to small home battery packs. Common residential storage involves battery-based inverters that can be used directly as backup power (e.g., a Tesla Powerwall that stores solar energy from rooftop panels) or an EV battery that can ship power back to the distribution grid and supply it to customers when needed. Distributed batteries could play an important role in supporting the reliability of distributed renewable energy and relieving strain on the transmission system.
4.4. Demand Response and Virtual Power Plants
Demand response involves consumers decreasing or shifting usage during peak periods in response to incentives provided by utilities, such as interruptible service agreements, time-of-use pricing, and critical peak pricing (OE n.d.). Initially, demand response options were largely limited to industrial customers who agreed to reduce electricity use when called upon, in exchange for compensation. More recently, it has become an option for residential and commercial customers as well, especially those using distributed generation resources, such as rooftop solar, EVs, and backup power. In the future, demand response could be used to shift electricity demand to low-cost hours to absorb renewable power that might otherwise be curtailed and use it to precool homes prior to peak heating hours or preheat water for later use (Wietelman et al. 2022). Distributed demand response is most effective when aggregated across customers. One house or business reducing its load may not do much for the grid, but when aggregated, demand response can shave a substantial portion off peak load, thereby serving as a virtual DER (Cummins Inc. 2021). The National Transmission Needs Study estimated that 5 percent of hours where energy is most expensive cover about 50 percent of the value of relieving transmission congestion (US DOE 2023). This concentration of value highlights a unique opportunity for technologies that can shift demand to relieve strain on transmission systems.
VPPs leverage a network of DERs and demand response systems to engage in active demand management. VPPs can include combinations of many kinds of physical assets—rooftop solar, DW energy, backup power generators, EVs, and batteries/inverters—that a utility or the system operator can control collectively to determine what resources to deliver to or take from the grid. VPP operators may also directly control customer devices, such as air conditioners, thermostats, and water heaters, remotely to help balance demand and supply on the system. In exchange, customers get lower electricity bills and/or financial incentives. However, establishing demand management programs and VPPs requires certain grid enhancements. Specifically, two-way communication technologies are needed to send and receive data and enable the remote control. Some examples include smart thermostats, advanced metering infrastructure (“smart meters”), and home-area-networks. Aggregation software is also crucial to bring together all individual sources effectively and create the coordination that enables a VPP. VPPs enable renewable integration and decarbonizing by supporting grid reliability and, as they are typically cheaper than building a new power plant, they also support affordability of electricity (Martin and Brehm 2023).
A virtue of battery storage systems, demand response, and VPPs is that they offer a greater degree of control to help balance the system that is not available from most individual DGs in isolation. To the extent that these systems can modify power draws from the grid to accommodate fluctuations in renewable supply, they can lessen the need to rely on large transmission interchanges between regions for that type of support. They could also reduce renewables curtailment more readily by absorbing excess generation to charge batteries or precool buildings during hours when power is abundant and cheap. For example, customers with storage systems and the right types of energy rate designs can use and discharge their batteries during hours with high net peak loads (and associated high prices) and charge them during other times when prices are low. VPPs are a relatively new grid solution, and it remains to be seen how effective they will be at smoothing consumption and relieving strain on generation and transmission systems.
4.5. Takeaways on Distributed Energy Resources
Overall, the benefits of DERs over building transmission to utility-scale centralized generation include faster installation time and avoided energy losses and transmission costs, which may lead to lower-cost energy supply. Because DERs are smaller and often built on an existing site (e.g., rooftop solar on houses), the deployment is much quicker, and they face lower regulatory hurdles for siting and interconnecting with the grid than new transmission and utility-scale projects. Unlike with GETs, those investing in renewable DERs (such as residential and commercial customers who can transact with the distribution utility) are the direct beneficiaries of the investment, due to the energy that they provide the owner.
Despite their reliability and other benefits, DERs have limitations and face important barriers. First, establishing a system of DERs still takes space and might be unpleasant to those living nearby because of the noise and unappealing visual aesthetics of some types of resources, such as DW (EPA n.d.). Careful planning and consultation with affected parties is important to siting and implementing larger community-scale projects, and greater reliance on quiet resources, such as batteries, could offset some concerns.
Second, technical challenges can be associated with adopting and connecting DERs to the grid. These barriers primarily relate to the local utility’s ability to do so safely and within regulatory boundaries. Specifically, utilities must ensure that the DER does not negatively impact grid reliability, stability, and safety (Kim and Fischer 2021). For example, the increased variability in electricity production from DERs could threaten grid stability. Furthermore, the lack of visibility of the growing number of behind-the-meter DERs challenges local and regional grid operators’ ability to do system planning and regulate power supply (Kim and Fischer 2021). The local distribution utility must be able to supply power to these households and businesses, including when outages or natural disasters occur, so the electricity demand of DER owners still needs to be taken into account in utility planning. Digital equipment interconnecting DERs to the grid and providing information to grid operators can help address the DER visibility challenge (Kim and Fischer 2021). Smart devices, such as smart meters, smart thermostats, electric heat pumps, and advanced inverters that adjust solar power generation allow DER owners to keep track of their resources in real time and utilities to monitor and control DER operations more efficiently. This digitalization can then be scaled up to aggregate DERs and serve as reliable VPPs.
Third, the increased reliance on electronic monitoring and digital communications raises important cybersecurity and data privacy concerns and challenges. The digitization and automated communication between DER owners and utilities or grid operators often uses the Internet, which is prone to hacking, ransomware, and other malicious attempts to destabilize the grid (Office of Cybersecurity, Energy Security, and Emergency Response and EERE 2022). This threat is enhanced when DER aggregators establish VPPs. Collectively, DER providers, equipment vendors, integrators, and operators need to work with the federal and state utility regulators and relevant government agencies to standardize robust procedures in cases of threats and attacks, prerequisites for DER approval, and technologies to ensure cybersecure systems and controls. Some best practices, including encryption, multifactor authentication, and skilled software security teams, need to be adapted to the specific DER deployment.
5. Microgrids
Decentralization entails not only deploying more DERs that are interconnected to the main grid but also establishing decentralized microgrids that can sustain themselves independently when needed. These localized power systems can operate autonomously and generate, store, and distribute energy to those within their networks, which may or may not be connected to the larger grid. Microgrids can provide electricity in remote/isolated areas where the transmission system is nonexistent or difficult to establish due to terrain and sustain critical infrastructure when natural disasters or other security threats lead to power outages in the main bulk power grid (Vine et al. 2017).
Microgrids typically consist of several different DERs and interconnected loads defined within a geographic boundary. Historically, the bulk of them relied on fossil fuel–fired CHP plants and power generators with reciprocating engines. However, as more and more renewable energy has been introduced, wind and solar have increasingly been used (Vine et al. 2017). As this transition occurs, smart microgrids (Figure 4) with energy storage systems, smart technologies, and software to manage demand-supply communications automatically and efficiently can be built. In the future, microgrids could incorporate emerging technologies, such as hydrogen fuel cells and even small modular nuclear reactors.
Figure 4. A Conceptual Microgrid
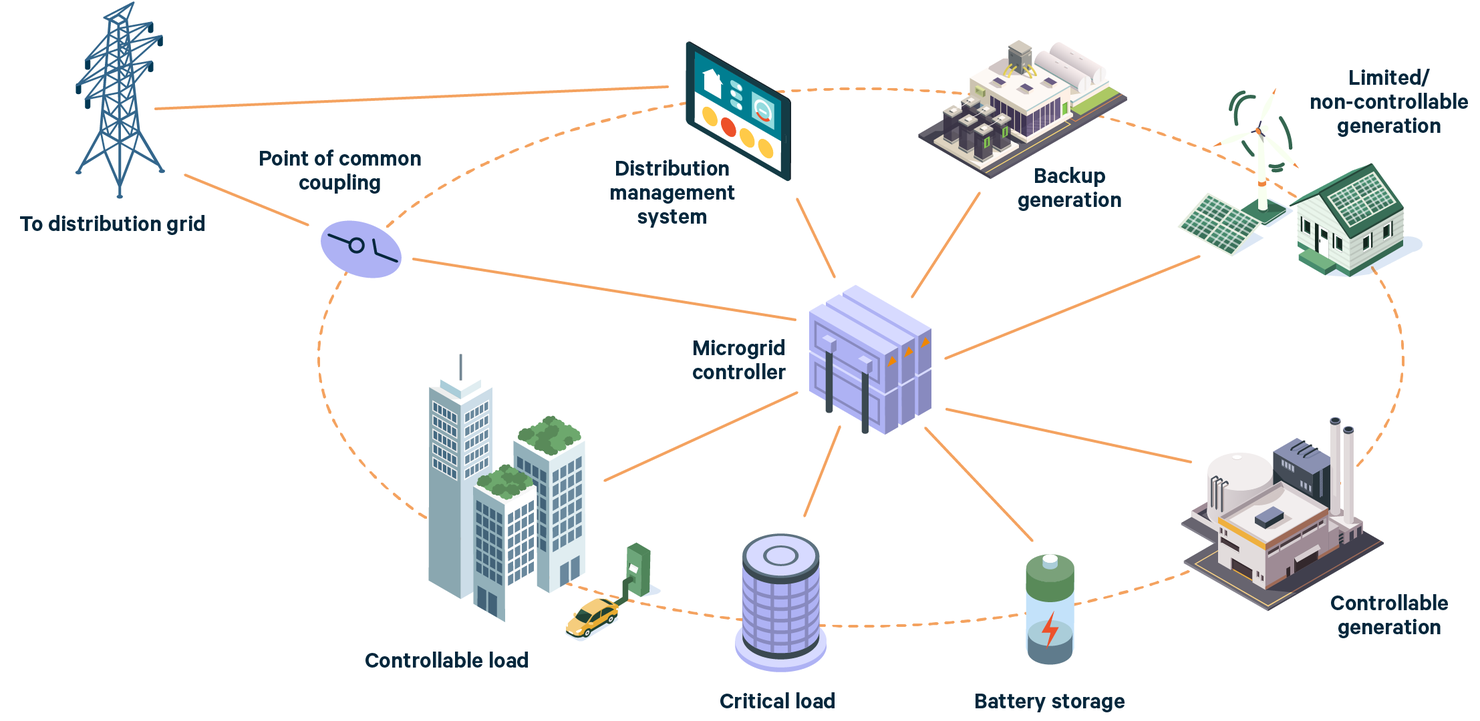
Microgrids vary by number of customers, the types of load and functions to address, connectivity to the main, larger grid, and ownership structure (Vine et al. 2017). Microgrids can serve a range of customers, from a single building to an entire neighborhood, and different types of loads—during emergencies or for day-to-day functioning. The extent of connection with the broader grid matters as well. If a microgrid is running in isolation, without the broader grid as a backup, it needs surplus generation (Vine et al. 2017). Finally, microgrids can be privately owned, utility-owned, publicly owned, or a combination of these. DOE provides a snapshot of US microgrid installations by primary application, technology, and size range. As of 2022, 687 sites have microgrids installed, and the total capacity is about 4.6 GW, https://doe.icfwebservices.com/microgrid (Excel data files) a miniscule share of the total US power generation capacity of 1,270 GW (Edison Electric Institute n.d.).
One of the most important advantages of microgrids is the resilience they offer against natural disaster events, such as hurricanes, wildfires, and snowstorms. Microgrids can sustain and continuously power university or industrial campuses, hospitals, or entire neighborhoods, when a natural or physical disaster causes outages on the main grid. They can also provide power to help re-energize the larger grid or essential power services to restoration crews for system recovery (Vine et al. 2017). For example, in North Carolina, a hybrid “Hybrid” refers to having more than one type of DER connected to the microgrid and using both conventional and zero-emissions resources. microgrid with 15 kW rooftop solar and a 3 MW diesel generator, along with a battery system, smart thermostats, and water heaters, was built to combat Ocracoke Island’s vulnerability to oceanic storms and complement the single submarine transmission line. In 2017, the islanded microgrid effectively maintained power when the transmission cable failed (Blair and De Martini 2020). The resilience services of a microgrid go above and beyond what basic transmission expansion can provide.
Microgrids can also play an important role in aggregating and interconnecting distributed generation and storage sources, making individual (and intermittent) DERs more reliable. For instance, on the Island of Kauai in Hawaii, the US Navy, in collaboration with the Kauai Island Utility Cooperative, independent power producer AES, and NREL, developed a project for the Pacific Missile Range Facility. This microgrid, comprised solely of 4 MW of solar with 70 MWh battery storage, makes the facility self-sufficient when transmission connections with the utility fail. The 100 percent clean microgrid also can black start itself without using backup diesel gensets, reducing the need for emitting generators (NREL 2021). This navy base microgrid has peripheral benefits to the community, and excess clean generation is available for customer use.
Microgrids using emissions-free DERs, coupled with proper coordination and intelligent control systems, can help manage local electricity demand and lead to cost savings for customers both on and off the microgrid. For their customers, microgrids facilitate peak-load shaving/shifting and demand response (see VPP section for details), thus reducing supply needs for peak demand and optimizing the deployment of connected DERs. This local response alleviates line congestion and leads to further cost savings via lower electricity prices. Additionally, microgrids can export power back to the utility and provide ancillary services, such as voltage control and frequency regulation (Konidena et al. 2020).
Microgrids can be a particularly important source of reliable power in remote locations with a single transmission line and a single point of vulnerability. Moreover, moving away from isolated diesel generators to a clean energy microgrid reduces exposure to fuel supply risks, price vulnerabilities, and adverse air quality impacts from diesel emissions (Konidena et al. 2020). Microgrids can keep emergency shelters operable during high heat or transmission and distribution outages. Clean energy microgrids also help sustain electricity service in remote disadvantaged communities where the supply is unreliable, including island nations, such as Puerto Rico and the American Samoa, and rural areas in the continental United States. Microgrids in a Native American Reservation in the Mojave Desert in California and the Ta’u island of the American Samoa are relevant case studies (Konidena et al. 2020). Building lines to provide similar resilience and reliability in remote areas could be prohibitively expensive. Community-owned microgrids could promote social equity among low-income, isolated, and Tribal communities by giving them more control over the use of their local natural resources and directly reaping the benefits of their energy systems.
Of course, microgrids are not a feasible solution to all transmission issues. Microgrids that rely predominantly on renewables, such as wind and solar, need abundant storage to be reliable and resilient. Storage is especially important for keeping critical facilities, such as hospitals, wastewater plants, and fire stations, running during extended bulk power grid outages and for remote microgrids that cannot fall back on the larger grid when wind and solar power generation is low. Batteries are the predominant solution, but maintaining sufficient battery storage systems for microgrid resiliency requires a large physical footprint and heavy batteries (Shahzad et al. 2023). Flywheels Flywheels are mechanical devices that transform electric power to mechanical power, in the form of kinetic energy, and store it for use. could be an alternative, but those are also bulky and expensive (Shahzad et al. 2023). Fucould be an alternative, but those are also bulky and expensive (Shahzad et al. 2023). Further research is needed on efficient and cost-effective energy storage systems to support microgrids and limit the need for connection to the larger grid.
Although microgrids can provide ancillary services to the main grid, distortions in voltage and frequency due to DERs, such as wind, solar, and battery storage, can create instability, causing power losses and overheated equipment (Shahzad et al. 2023). Advanced control technologies and monitoring systems may be needed to address these challenges. The increased deployment of smart microgrids will increase the need for greater coordination and collaboration through secure data and information sharing among stakeholders, including regulators, utilities, and distributed energy producers, such as residential customers and local communities. As with individual DERs, cybersecurity issues related to communications and control systems are also an issue and will require protection systems and best practices.
It is also not clear how easy it is for microgrid projects to get financing. Each project is different based on the geography, weather, markets, load requirements, and microgrids integrate several different energy sources and technologies, making it complex and challenging for investors to evaluate them from a financing perspective (Vine et al. 2017). Differences in policies, including tax incentives and regulations, around siting and building infrastructure for wind versus solar can complicate project evaluation. Moreover, microgrids have high up-front costs, and the perceived risks of different technologies due to their complexity and unfamiliarity make it challenging to attract investment (Vine et al. 2017). Additional legal and regulatory issues exist, such as laws limiting who can supply electricity retail services and electricity sales by consumers, as well as export restrictions. Financing challenges also vary by ownership type. Utilities can draw on ratepayer funds, and privately owned microgrids that typically rely on debt financing may be disadvantaged in terms of revenue assurance (Vine et al. 2017). Community-owned microgrids have been able to rely on federal and state grants. The variety of potential revenue streams, including renewable credits and some customers’ demand for highly reliable power, complicate project evaluation and pose hurdles in convincing investors to fund microgrids instead of a simple solar installation or wind farm.
Regulations can also limit microgrid development. Rules, prompted in part by concerns about cost allocation and cross subsidies, may limit or forbid a utility’s ownership of distributed resources, reducing its involvement in microgrids. State laws that fail to distinguish between distribution services provided by the bulk power grid and microgrids can prove too costly for nonutility microgrid owners. Last, as state laws typically grant rights of way over public streets to regulated distribution utilities, a microgrid wanting to serve a small area with multiple customers may be unable to do so or need to take on additional responsibilities of a public utility (Vine et al. 2017).
Policies that suppress microgrid adoption, particularly those that benefit both direct customers and distribution utilities, could increase social inequity as more affluent customers adopt individual DERs and reduce their reliance on the bulk grid. Alternatively, absent efforts to make them more accessible and affordable, the benefits of microgrids may exclusively flow to those (individuals or communities) who can afford the high costs of development (Shahzad et al. 2023). Therefore, a critical aspect of implementing microgrids more broadly is providing additional financial and technical support to disadvantaged communities through federal programs, such as the Justice40 initiative (Office of Economic Impact and Diversity n.d.). Educational outreach can help to relay information on the economic and environmental benefits of a microgrid deployment and on programs such as the IIJA, tax credits, and Renewable Energy Credits that can support development (NARUC and NASEO 2023).
5.1. Takeaways on Microgrids
Overall, microgrids can introduce important efficiencies by avoiding transmission losses and the additional generation needed to cover them (Vine et al. 2017). Microgrids can also be deployed more quickly than typical transmission planning and construction (NARUC and NASEO 2023). Thus, in certain situations, microgrids could substitute for new transmission. The opportunities are most pronounced in rural and remote locations and areas where lines are vulnerable to extreme weather and therefore unreliable. Additionally, microgrids could also serve places where demand is highly correlated with the availability of clean electricity (e.g., high demand during heat waves and high solar supply). However, because microgrids, by definition, serve small pockets of customers, involve DERS with high levelized costs, and have multiple financing, legal, and regulatory complexities, they will not fully substitute for the overall need for additional transmission. More research is needed to identify which places, regions, and communities would be best served by microgrids and where utility–private joint ownership, along with government support, could be an efficient microgrid business model. See Blair and De Martini (2020) for details on various utility–private joint ownership microgrids that have developed in the United States and how this model could take advantage of the financial and institutional capabilities of various stakeholders to meet the needs of the local community and businesses.
6. Conclusion
Significant barriers exist to planning, siting, permitting, and building new transmission lines, and the process can take several years to complete. The urgent need to expand the capabilities of the electric power system and integrate more renewable and clean energy prompts us to investigate alternative investments and technologies that can enhance the capabilities of the existing system in the near term and at least partially ameliorate the need for new investment. This report explores three broad categories: GETs, DERs, and microgrids. Each approach has its strengths and weaknesses, and a coordinated effort among policymakers, regulators, utilities, technology developers, investors, and consumers is needed to assess and implement the most efficient and cost-effective complements and alternatives to transmission expansion. Moreover, although these investments may all somewhat relieve the demand for expansion, they may only act as substitutes in a specific set of circumstances. Generally, our analysis suggests the following areas of opportunity:
- GETs: GETs provide a relatively low-cost approach to support and enhance a system’s capabilities by maximizing line efficiency and minimizing congestion. This translates to lower electricity costs by way of reducing congestion costs. In particular, GETs may offer enhanced power deliverability in areas with an underused, robust physical system. High-density areas with many lines may benefit from upgrades to the system that allow more power to flow and enable it to withstand more variable resources. However, incentives need to be modified to adopt these software and hardware technologies, and more research is needed on making them more accurate. Overall, GETs can defer the need for new transmission and generation but are limited by the system’s footprint and owner incentives.
- DERs: DERs can play a critical role integrating renewable energy into the grid. Different technologies have different benefits, but each contributes to more affordable electricity and reduces the burden on the transmission system by offering an opportunity to co-locate energy demand with supply from smaller generation systems, which can defer or reduce the need to connect large utility-scale generation projects to centers of demand (and avoid permitting and siting challenges for those lines). However, decarbonization of the electricity sector by deploying clean or zero-emissions DERs, overcoming regulatory barriers, and addressing cybersecurity and data privacy concerns is crucial.
- Demand response and VPPs: Demand response has long been considered a critical element of electricity affordability in the context of a decarbonized grid. The ability to avoid high peak prices for generation is extremely valuable. Demand response and VPP capabilities could also relieve demand on the system by lowering or meeting peak demand that would otherwise require connecting more generation sources to the bulk power system. However, more evidence is needed on exactly how much demand, and from which sources, can be shifted using these tools.
- Microgrids: Microgrids, composed of various DERs, largely offer reliability and resiliency benefits to electricity users that do not want to or cannot rely on the bulk power system. This is especially true for remote areas, islands, and disadvantaged communities where transmission infrastructure is weak and more vulnerable, where additional transmission investment may offer inadequate solutions, be less effective, or be more costly than creating microgrids with self-serving generation that can operate independently. However, the financial, legal, and regulatory hurdles to microgrids may prevent them from being deployed effectively and render them difficult to implement. Hence, more research is needed on where they can deliver the most value.
Our analysis also reveals several areas where more research is needed to determine the value of these investments. For example, where could GETs deliver the most value on the existing system and reduce the need for new transmission and generation investments? Furthermore, analysis could reveal whether additional incentives for DERs could be more cost-effective than building new lines to connect utility-scale renewables to areas of high demand. Finally, research is needed to determine how microgrids may be sited to maximize their impacts on overall grid reliability, rather than just offering resilience to their direct connections. Investments in new transmission lines will undoubtedly play a role in grid decarbonization, but the alternative investments explored in this report may ease the regulatory and financial burdens of building new lines by offering faster and/or lower-cost ways to increase the clean capacity, affordability, and reliability of our grid.